Preparing for the MCAT necessitates a profound comprehension of neuron membrane potentials, essential for mastering Organ Systems. Understanding the mechanisms of action potentials and resting potentials, as well as ion channel functions, equips you with crucial insights into neuronal communication, vital for excelling on the MCAT and understanding complex neural functions.
Learning Objective
In studying “Neuron Membrane Potentials” for the MCAT, you should develop an understanding of the electrical properties of neurons, including the generation and propagation of action potentials and the establishment of resting membrane potentials. Explore the roles of ion channels, pumps, and the selective permeability of the neuronal membrane. Evaluate how changes in membrane potentials facilitate neuronal communication and signal integration. Additionally, understand how these electrical phenomena are foundational to neural circuit function and behavior. Apply this knowledge to interpreting data from electrophysiological recordings and solving MCAT practice questions on neuronal behavior and pharmacological impacts on nerve function.
Introduction to Neuron Membrane Potentials
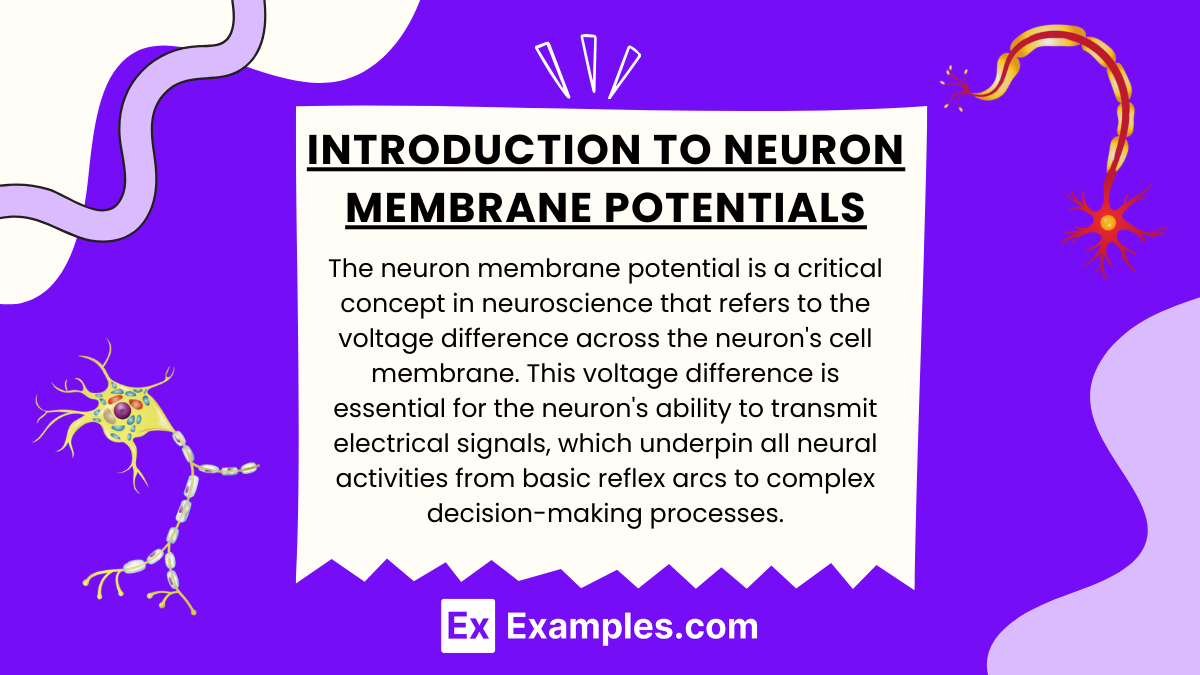
The neuron membrane potential is a critical concept in neuroscience that refers to the voltage difference across the neuron’s cell membrane. This voltage difference is essential for the neuron’s ability to transmit electrical signals, which underpin all neural activities from basic reflex arcs to complex decision-making processes. Here’s a deeper look into the definition and underlying concepts:
Membrane Potential
The membrane potential of a neuron is the electrical potential difference (voltage) across its cell membrane, determined by the distribution of ions between the inside and outside of the neuron. This potential can vary between different stages of neuronal activity, generally existing as either the resting membrane potential or the action potential.
Conceptual Understanding
1. Resting Membrane Potential
- Nature: It is the baseline state of the neuron when it is not transmitting signals. Typically, this is about -70 millivolts (mV), meaning the inside of the neuron is 70 mV less positive than the outside.
- Maintenance: It is maintained primarily through the action of ion pumps and ion channels. The most notable mechanism is the sodium-potassium pump, which uses ATP energy to exchange three sodium ions out of the neuron for two potassium ions into the neuron. This activity, combined with different permeabilities of the ion channels for sodium and potassium, maintains the negative interior.
2. Action Potential
- Triggering: When a neuron receives a sufficient external signal (a stimulus that brings the membrane potential to a threshold level, typically around -55 mV), the neuron’s membrane potential rapidly rises and falls.
- Process:
- Depolarization: Voltage-gated sodium channels open, allowing sodium ions to flow into the neuron, making the inside less negative (more positive).
- Repolarization: Soon after the peak, sodium channels close and potassium channels open, allowing potassium ions to flow out, returning the potential towards the negative.
- Hyperpolarization: Sometimes the efflux of potassium is excessive, causing the potential to become even more negative than the resting potential temporarily.
3. Ion Channels and Pumps
- Ion Channels: These are specific for ions and can be gated (open or close in response to stimuli) or non-gated. They allow ions to move according to concentration gradients, playing key roles during action potentials.
- Ion Pumps: These actively transport ions against their concentration gradients using energy, crucial for maintaining the resting potential and readying the neuron for another action potential.
Importance of Membrane Potentials
Understanding membrane potentials is crucial for several reasons:
- Signal Transmission: They enable the transmission of electrical signals over distances within the brain and throughout the nervous system.
- Neural Communication: Changes in membrane potentials at synapses affect neurotransmitter release, influencing how neurons communicate and ultimately process information.
- Basis for Medical Applications: Knowledge of how membrane potentials work helps in understanding and treating neurological diseases and conditions that affect brain function.
Generation of Membrane Potentials
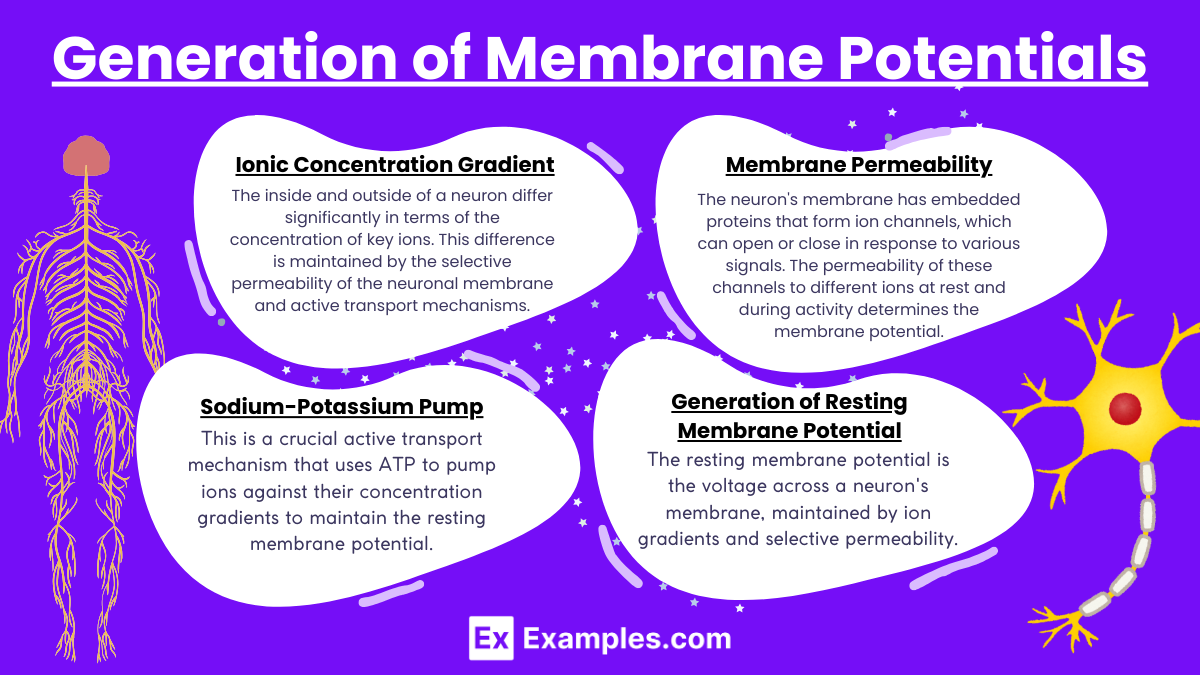
The generation of membrane potentials in neurons is a dynamic process that relies on the interplay of ion concentrations and membrane permeability. This process is crucial for the excitability of neurons, enabling them to initiate and propagate electrical signals. Here’s how membrane potentials are generated:
1. Ionic Concentration Gradient
The inside and outside of a neuron differ significantly in terms of the concentration of key ions. This difference is maintained by the selective permeability of the neuronal membrane and active transport mechanisms.
- Inside the Neuron: High concentrations of potassium ions (K⁺) and negatively charged proteins (anions).
- Outside the Neuron: High concentrations of sodium ions (Na⁺) and chloride ions (Cl⁻).
2. Membrane Permeability
The neuron’s membrane has embedded proteins that form ion channels, which can open or close in response to various signals. The permeability of these channels to different ions at rest and during activity determines the membrane potential.
- Resting State: Potassium channels are predominantly more open compared to sodium channels, making the membrane more permeable to potassium. Sodium channels, while present, are mostly closed at rest.
3. Sodium-Potassium Pump
This is a crucial active transport mechanism that uses ATP to pump ions against their concentration gradients to maintain the resting membrane potential:
- Pumps three Na⁺ ions out of the cell.
- Pumps two K⁺ ions into the cell. This results in a net loss of positive charge from the neuron, contributing to the negative resting membrane potential.
4. Generation of Resting Membrane Potential
The resting membrane potential is the voltage across a neuron’s membrane, maintained by ion gradients and selective permeability.
- Efflux of Potassium: Due to the concentration gradient and the membrane’s permeability, potassium ions tend to move out of the neuron, carrying positive charge with them. This movement creates a more negative charge inside compared to outside.
- Limited Sodium Influx: While some sodium channels are open, the relative impermeability to sodium at rest means only a little sodium enters the neuron compared to the potassium leaving.
Synaptic Transmission and Integration
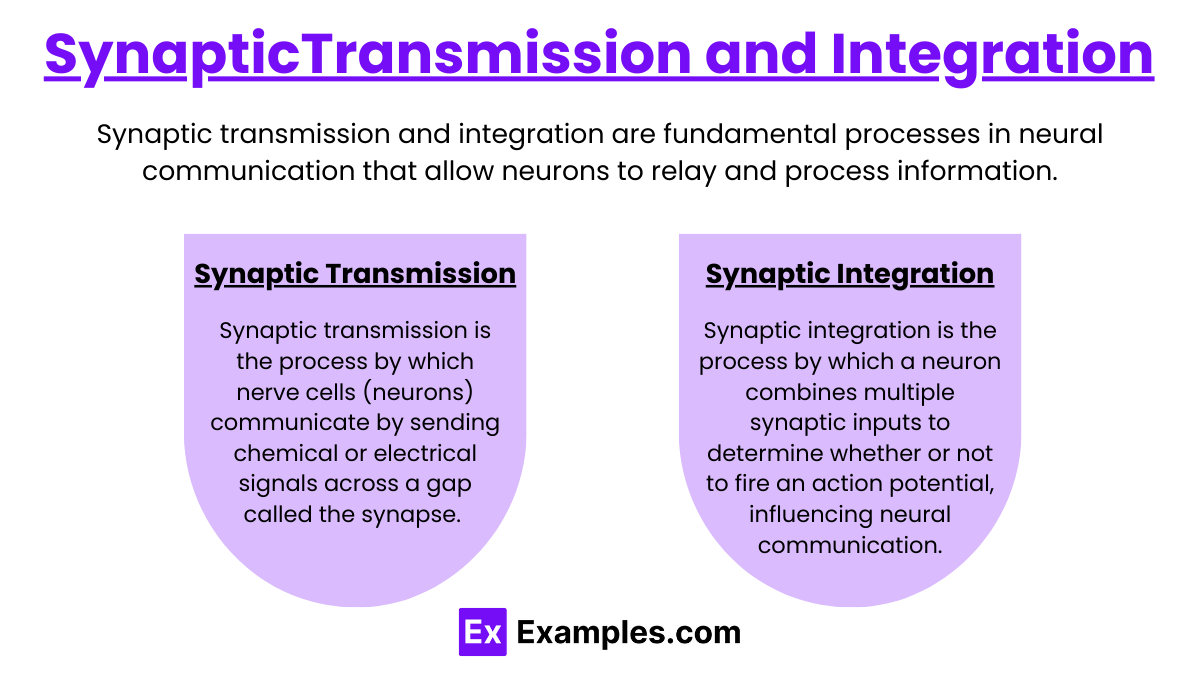
Synaptic transmission and integration are fundamental processes in neural communication that allow neurons to relay and process information. Here’s a condensed overview:
Synaptic Transmission
Synaptic transmission is the process by which nerve cells (neurons) communicate by sending chemical or electrical signals across a gap called the synapse. which can be:
- Electrical Synapses: Direct electrical currents flow between neurons through gap junctions, facilitating fast synchronization.
- Chemical Synapses: Neurotransmitters are released from the presynaptic neuron into the synaptic cleft, where they bind to receptors on the postsynaptic neuron, triggering excitatory or inhibitory responses depending on the neurotransmitter type.
Synaptic Integration
Synaptic integration is the process by which a neuron combines multiple synaptic inputs to determine whether or not to fire an action potential, influencing neural communication. Key mechanisms include:
- Temporal Summation: Rapid, successive signals at a single synapse can cumulatively trigger an action potential.
- Spatial Summation: Simultaneous signals from multiple synapses can combine to influence the postsynaptic response.
- Postsynaptic Potentials:
- EPSPs (Excitatory Postsynaptic Potentials): Make the neuron more likely to fire by depolarizing it.
- IPSPs (Inhibitory Postsynaptic Potentials): Make the neuron less likely to fire by hyperpolarizing it.
- Modulation: Neuromodulators adjust synaptic strength, affecting neurotransmitter release or postsynaptic response, which impacts neural circuit function and responsiveness.
Examples
Example 1:Generation of an Action Potential
- A neuron in the brain receives a stimulus from a sensory receptor.
- If the stimulus is strong enough to cause the neuron’s membrane potential to reach the threshold, voltage-gated sodium channels open, sodium ions rush into the neuron, causing rapid depolarization that initiates an action potential.
Example 2:Role of the Sodium-Potassium Pump
- Maintaining the resting potential after repeated neuronal firing.
- The sodium-potassium pump actively transports 3 sodium ions out of and 2 potassium ions into the neuron, using ATP for energy. This action helps restore and maintain the resting membrane potential, ensuring the neuron is ready for the next action potential.
Example 3:Effects of Local Anesthetics
- Application of lidocaine to block pain signals.
- Lidocaine stabilizes the neuronal membrane by blocking voltage-gated sodium channels. This prevents the initiation and propagation of action potentials along sensory nerves, effectively reducing pain perception.
Example 4:Summation Leading to Neuronal Firing
- A neuron in the spinal cord receives multiple synaptic inputs.
- Temporal summation occurs when several high-frequency signals arrive in quick succession at a single synapse, cumulatively depolarizing the neuron enough to reach the threshold. Spatial summation occurs when inputs from multiple locations combine at the same time to trigger an action potential.
Example 5:Hyperkalemia Affecting Heart Muscle Cells
- Elevated blood potassium levels interfere with cardiac function.
- High extracellular potassium reduces the gradient across the membrane of heart muscle cells, which can diminish the cells’ ability to repolarize and prepare for subsequent muscle contractions. This can lead to cardiac arrhythmias and requires immediate medical attention.
Practice Questions
Question 1:
What primarily maintains the negative resting membrane potential of a neuron?
A) The influx of sodium ions through voltage-gated channels
B) The efflux of potassium ions through leak channels
C) The influx of calcium ions
D) The active transport of glucose into the neuron
Answer: B) The efflux of potassium ions through leak channels
Explanation:
The negative resting membrane potential of a neuron is primarily maintained by the efflux of potassium ions through leak channels. Potassium ions tend to diffuse out of the neuron due to a higher internal concentration, and their movement out of the cell contributes to the inside becoming more negative relative to the outside. This efflux is more significant in setting the resting potential than the influx of other ions.
Question 2:
During an action potential, what event directly follows the rapid influx of sodium ions into the neuron?
A) Potassium ions flow out of the neuron
B) Sodium ions are actively pumped out of the neuron
C) Calcium ions enter the neuron
D) Glucose is metabolized within the neuron
Answer: A) Potassium ions flow out of the neuron
Explanation:
Following the rapid influx of sodium ions into the neuron during depolarization, potassium channels open, and potassium ions flow out of the neuron. This efflux of potassium helps to repolarize the membrane, restoring the negative internal environment of the neuron and leading to the falling phase of the action potential.
Question 3:
Which of the following drugs would likely increase the duration of an action potential in a nerve cell?
A) A drug that blocks voltage-gated sodium channels
B) A drug that blocks voltage-gated potassium channels
C) A drug that increases the activity of sodium-potassium pumps
D) A drug that inhibits the release of neurotransmitters
Answer: B) A drug that blocks voltage-gated potassium channels
Explanation:
A drug that blocks voltage-gated potassium channels would likely increase the duration of an action potential. By preventing potassium from leaving the neuron during the repolarization phase, the action potential is prolonged, delaying the return of the membrane potential to its resting state. This effect can be significant in various clinical settings, such as in the treatment of certain cardiac arrhythmias.