Preparing for the MCAT requires a thorough understanding of thermodynamics, a foundational concept in physics and chemistry. Mastery of energy transfer, entropy, and the laws of thermodynamics is essential. This knowledge provides insights into biochemical reactions, cellular metabolism, and energy balance, crucial for achieving a high MCAT score.
Learning Objective
In studying “Thermodynamics” for the MCAT, you should aim to understand the fundamental principles governing energy transfer, including the laws of thermodynamics. Analyze concepts such as enthalpy, entropy, and Gibbs free energy, and how they apply to spontaneous and non-spontaneous reactions. Evaluate the role of thermodynamic principles in biochemical processes, including cellular respiration and ATP hydrolysis. Additionally, explore how temperature, pressure, and volume affect reaction equilibrium and energy dynamics. Apply this knowledge to interpret thermodynamic data and reaction scenarios in MCAT practice passages, enhancing your ability to analyze energy changes and reaction feasibility.
Fundamental Laws of Thermodynamics
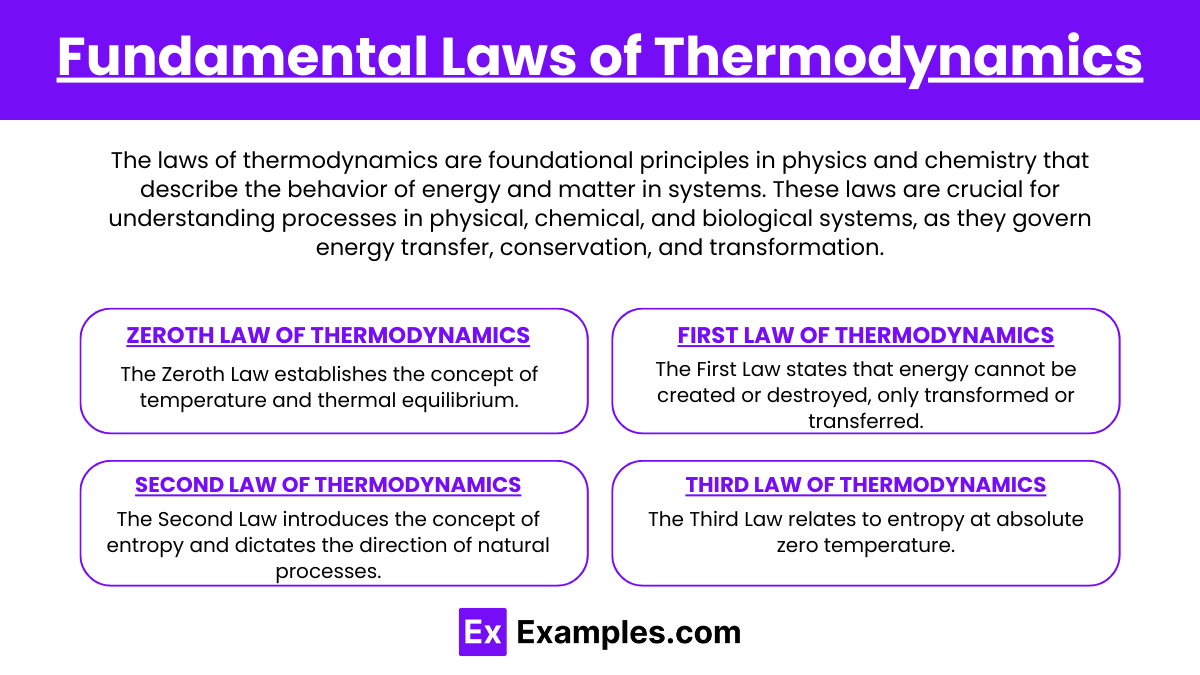
The laws of thermodynamics are foundational principles in physics and chemistry that describe the behavior of energy and matter in systems. These laws are crucial for understanding processes in physical, chemical, and biological systems, as they govern energy transfer, conservation, and transformation.
Zeroth Law of Thermodynamics:
The Zeroth Law establishes the concept of temperature and thermal equilibrium.
- Statement: If two systems, A and B, are each in thermal equilibrium with a third system, C, then A and B are also in thermal equilibrium with each other.
- Implication: This law forms the basis for measuring temperature, as it implies that all three systems share the same temperature if they are in thermal equilibrium.
First Law of Thermodynamics (Law of Energy Conservation):
The First Law states that energy cannot be created or destroyed, only transformed or transferred.
- Statement: The change in internal energy of a system (ΔU) is equal to the heat (Q) added to the system minus the work (W) done by the system: ΔU=Q−W.
- Implication: This law emphasizes the conservation of energy. In any process, the total energy remains constant, with energy changing form (e.g., from heat to work or vice versa).
Second Law of Thermodynamics:
The Second Law introduces the concept of entropy and dictates the direction of natural processes.
- Statement: In any energy transfer or transformation, the total entropy (disorder) of an isolated system will either increase or remain the same. It will never decrease.
- Implication: This law implies that natural processes tend toward a state of maximum entropy. It also explains why certain processes are irreversible, like heat spontaneously flowing from a hot object to a cold one.
Third Law of Thermodynamics:
The Third Law relates to entropy at absolute zero temperature.
- Statement: As the temperature of a system approaches absolute zero, the entropy of a perfectly ordered, pure crystalline structure approaches zero.
- Implication: This law implies that it is impossible to reach absolute zero in practice, as removing all entropy from a system becomes increasingly difficult as temperature decreases.
Key Thermodynamic Concepts
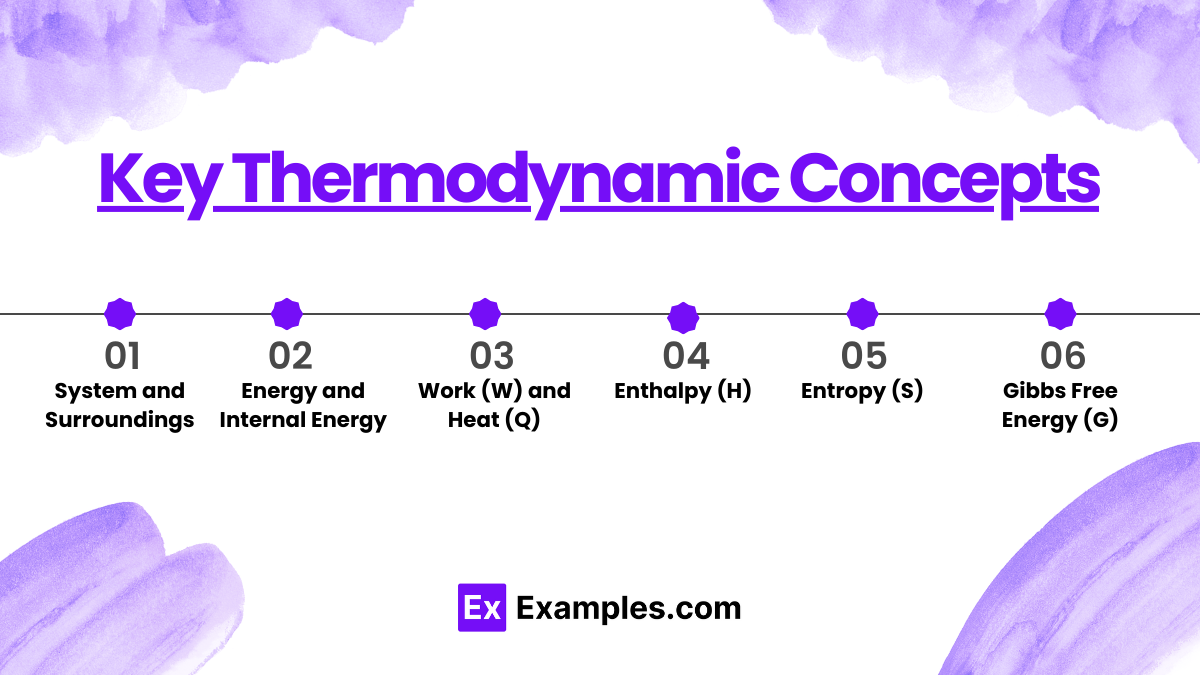
Thermodynamics is the study of energy, heat, and work within physical systems. Here are some essential concepts that form the basis of thermodynamic principles and their applications:
1. System and Surroundings
- System: The part of the universe being studied (e.g., a gas in a container).
- Surroundings: Everything outside the system that can interact with it.
- Types of Systems:
- Isolated System: No exchange of energy or matter with the surroundings.
- Closed System: Exchanges energy (heat or work) but not matter with surroundings.
- Open System: Exchanges both energy and matter with surroundings.
2. Energy and Internal Energy
- Energy: The capacity to do work or produce heat, existing in various forms such as kinetic, potential, chemical, and thermal energy.
- Internal Energy (U): The total energy contained within a system, influenced by molecular motion and chemical bonds. Internal energy changes when heat is added or removed, or when work is done on or by the system.
3. Work (W) and Heat (Q)
- Work: Energy transfer that occurs when an external force moves an object or changes the volume of a system (e.g., gas expansion).
- Heat: Energy transfer due to a temperature difference between the system and surroundings, flowing from high to low temperature.
- Relation to Energy: According to the First Law of Thermodynamics, changes in internal energy are due to work done and heat transferred: ΔU=Q−W.
4. Enthalpy (H)
- Enthalpy is a measure of total heat content in a system at constant pressure, represented as H=U+PV (where P is pressure, V is volume, and U is internal energy).
- Implication: Changes in enthalpy (ΔH) are useful for understanding heat exchange in chemical reactions under constant pressure, such as in exothermic or endothermic reactions.
5. Entropy (S)
- Entropy is a measure of disorder or randomness in a system. Higher entropy indicates greater disorder.
- Implication: According to the Second Law of Thermodynamics, entropy in an isolated system tends to increase over time, leading to irreversible processes and a natural direction for spontaneous changes.
6. Gibbs Free Energy (G)
- Gibbs Free Energy, G=H−TS (where T is temperature, S is entropy, and H is enthalpy), represents the amount of energy available to do useful work at constant temperature and pressure.
- Implication: Changes in Gibbs Free Energy (ΔG) determine whether a reaction or process is spontaneous:
- ΔG<0: The process is spontaneous (favorable).
- ΔG>0: The process is non-spontaneous (unfavorable).
- ΔG=0: The system is in equilibrium.
Effect of Temperature, Pressure, and Volume on Reactions
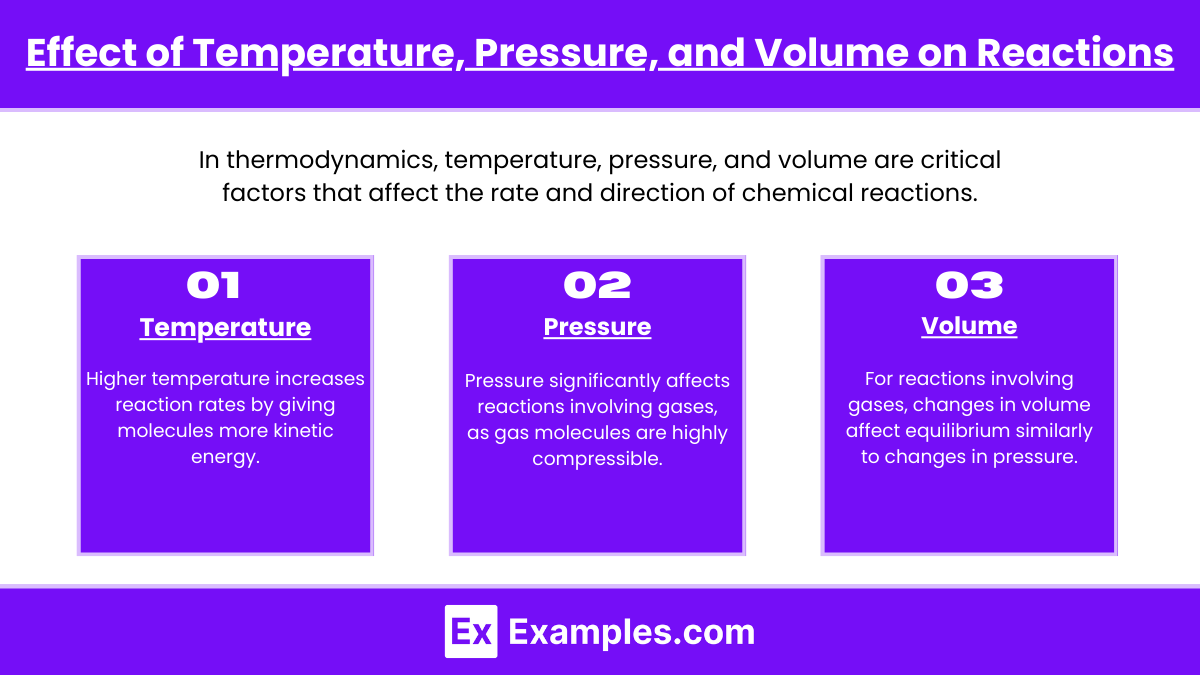
In thermodynamics, temperature, pressure, and volume are critical factors that affect the rate and direction of chemical reactions. Understanding how these variables influence reactions helps predict outcomes, control reaction conditions, and optimize processes in both industrial and biological systems.
- Temperature:
- Reaction Rate: Higher temperature increases reaction rates by giving molecules more kinetic energy.
- Equilibrium Shift:
- Endothermic Reactions: Higher temperature shifts equilibrium toward products.
- Exothermic Reactions: Higher temperature shifts equilibrium toward reactants.
- Activation Energy: More molecules can overcome activation energy at higher temperatures.
- Pressure (primarily affects gases):
- Increasing Pressure: Favors the side with fewer gas molecules, shifting equilibrium accordingly.
- Decreasing Pressure: Favors the side with more gas molecules.
- Ideal Gas Law: PV=nRT links pressure, volume, and temperature for gases.
- Volume (primarily affects gases):
- Decreasing Volume: Increases pressure, favoring the side with fewer gas molecules.
- Increasing Volume: Decreases pressure, favoring the side with more gas molecules.
- Concentration Changes: Lower volume increases gas concentration, potentially shifting equilibrium.
Applications and Problem Solving in Thermodynamics
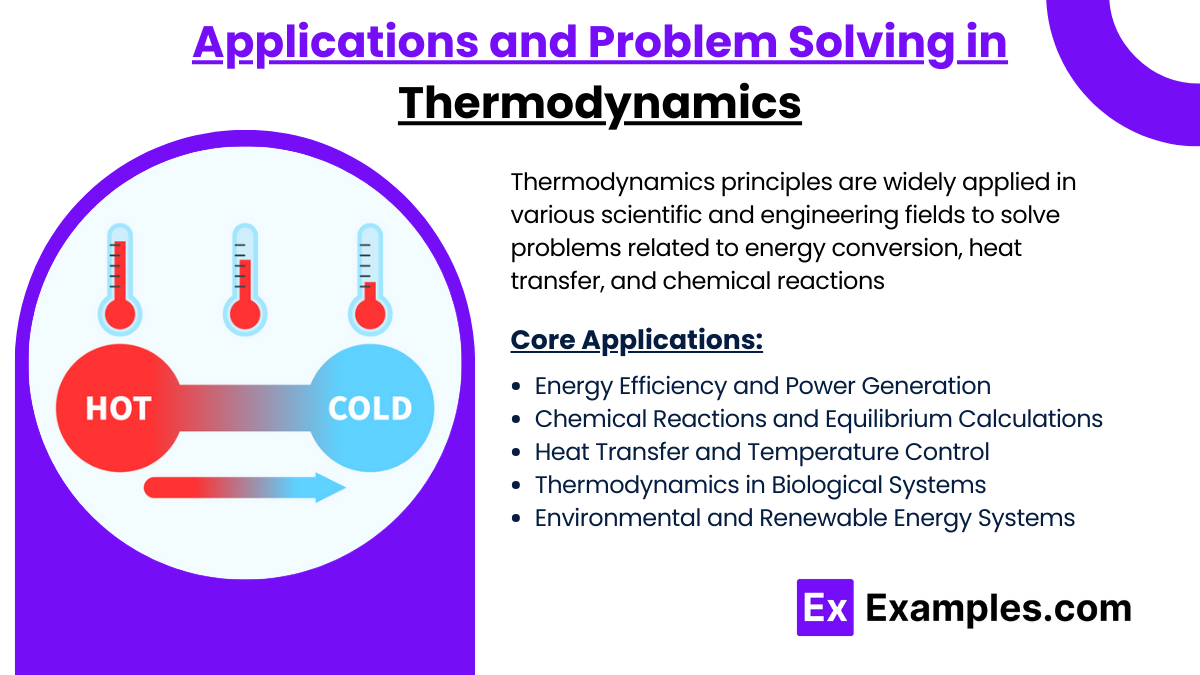
Thermodynamics principles are widely applied in various scientific and engineering fields to solve problems related to energy conversion, heat transfer, and chemical reactions. Here are some core applications and problem-solving approaches in thermodynamics:
1. Energy Efficiency and Power Generation
- Application: Thermodynamics is crucial in designing efficient power systems, such as engines, turbines, and refrigerators, where maximizing energy conversion and minimizing waste heat are key.
- Problem Solving: Use the First Law of Thermodynamics to calculate energy input, output, and losses in cycles (e.g., Carnot cycle) to determine system efficiency.
2. Chemical Reactions and Equilibrium Calculations
- Application: Thermodynamic principles are essential for predicting reaction spontaneity, equilibrium position, and yield in chemical processes.
- Problem Solving: Calculate Gibbs Free Energy (ΔG) using enthalpy (ΔH), entropy (ΔS), and temperature to determine whether a reaction is spontaneous (ΔG<0) or requires energy (ΔG>0). Adjust temperature or pressure to optimize reactions based on Le Chatelier’s Principle.
3. Heat Transfer and Temperature Control
- Application: Heat transfer calculations are important in managing temperatures in buildings, industrial processes, and electronic devices.
- Problem Solving: Apply heat transfer equations (conduction, convection, and radiation) to calculate heat flow, using specific heat and heat capacity to determine how much energy is needed to change the temperature of materials.
4. Thermodynamics in Biological Systems
- Application: Thermodynamics helps explain energy usage, metabolism, and heat regulation in living organisms.
- Problem Solving: Calculate energy conversion efficiency in biological processes, like ATP production, or use enthalpy and entropy changes to understand metabolic pathways.
5. Environmental and Renewable Energy Systems
- Application: Thermodynamics underpins the design of solar panels, wind turbines, and fuel cells, focusing on maximizing energy conversion from renewable sources.
- Problem Solving: Use efficiency calculations to compare energy outputs of different renewable systems and apply enthalpy and Gibbs Free Energy equations to analyze fuel cell performance.
Examples
Examples 1: First Law of Thermodynamics in Cellular Respiration
- During cellular respiration, the energy stored in glucose is converted into ATP, with some energy lost as heat. This illustrates the First Law of Thermodynamics, where energy is conserved but transformed between chemical, mechanical, and thermal forms within the cell.
Examples 2: Gibbs Free Energy and ATP Hydrolysis
- ATP hydrolysis has a negative Gibbs free energy (ΔG<0), making it a spontaneous reaction that releases energy. This energy drives various cellular processes, such as muscle contraction, by coupling with non-spontaneous reactions.
Examples 3: Entropy in Protein Folding
- When a protein folds into its functional 3D structure, the system’s entropy decreases as the polypeptide chain organizes. However, the overall entropy (protein plus surrounding water) increases, illustrating the Second Law of Thermodynamics, as the process is energetically favorable.
Examples 4: Temperature Dependence of Enzyme Activity
- Enzymes function optimally at specific temperatures; for instance, human enzymes work best around 37°C. Increasing temperature generally speeds up reactions until a point where the enzyme denatures, highlighting the temperature dependence of reaction rates.
Examples 5: Le Chatelier’s Principle in Oxygen Transport
- In the lungs, high oxygen partial pressure shifts the equilibrium towards oxyhemoglobin formation. In tissues with low oxygen, equilibrium shifts towards releasing oxygen from hemoglobin, demonstrating how pressure influences equilibrium in biological systems.
Practice Questions:
Question 1
Which of the following describes a spontaneous reaction?
A) ΔG > 0
B) ΔG = 0
C) ΔG < 0
D) ΔH = 0
Answer: C) ΔG < 0
Explanation:
A spontaneous reaction occurs when the Gibbs free energy change (ΔG) is negative, meaning ΔG < 0. This indicates that the reaction releases free energy, which can occur under conditions where enthalpy (ΔH) is negative or entropy (ΔS) increases. If ΔG > 0, the reaction is non-spontaneous, and if ΔG = 0, the system is at equilibrium.
Question 2
What happens to the entropy of a system when a protein folds into its functional structure?
A) Entropy increases
B) Entropy decreases
C) Entropy remains constant
D) Entropy becomes zero
Answer: B) Entropy decreases
Explanation:
When a protein folds, it goes from an unfolded, more random state to a structured, organized state, which reduces the system’s entropy. However, the surrounding water molecules experience an increase in entropy, making the overall process favorable in terms of the Second Law of Thermodynamics.
Question 3
According to the First Law of Thermodynamics, which of the following statements is true?
A) Energy can be created and destroyed.
B) Energy can only be transferred, not transformed.
C) Energy in an isolated system is constant.
D) Entropy in a closed system always decreases.
Answer: C) Energy in an isolated system is constant.
Explanation:
The First Law of Thermodynamics states that energy cannot be created or destroyed, only transferred or transformed. In an isolated system, the total energy remains constant. This principle is fundamental to all energy transformations, including biochemical reactions. The other options misstate key thermodynamic principles, particularly regarding energy conservation and entropy.